Nature provides a huge set of biological catalysts (biocatalysts) able to activate otherwise unreactive compounds and catalyze their conversions with an excellent combination of energy efficiency, turnover frequency and selectivity unmatched by synthetic counterparts. Many biocatalysts can catalyze redox reactions, and replacing their natural electron donor or acceptor by an electrode allows for precise control over the reaction direction (oxidation/reduction), the driving force (overpotential) and the catalytic rates the system is exposed to. Therefore, electrochemical biocatalysis is investigated to address energy storage, production of sustainable fuels/chemicals and the precise sensing of analytes.1 To avoid sluggish diffusion processes and excess demand of the biocatalysts, they are typically immobilized onto electrode surfaces forming redox active films. The most prominent types of film-forming biocatalysts are either isolated enzymes or microbial cells.2 The former exhibit high energy efficiency, often even allowing for reversible electrocatalysis and high turnover frequencies (e.g. for the conversion of H2 or CO2) making them highly attractive in the field of electrochemical energy conversion.3,4 Furthermore, the excellent selectivity of redox enzymes already led to a variety of different enzyme-based electrochemical sensors.5 However, many isolated enzymes degrade rapidly in the presence of exaggerated pH values, high electrode potentials, light irradiation or oxygen. Microbial cell catalysis instead offers more stable electrochemical biocatalysis due to the self-repairing and self-replicating capability of living cells.6,7 Moreover, microbial cells are more promiscuous in their substrates and can catalyze a variety of different reactions or even complex sequences of reactions.8 However, these advantages come at the expense of a high catalyst footprint, potentially slow electron transfer9 or diffusion processes over the cell membrane and a lowered product selectivity. Thus, current research focuses on an in-depth understanding of the complex processes in microbial catalysis10 and on increasing the operational stability of redox enzymes. Due to their complementary advantages, both, enzymatic and microbial electrochemical biocatalysis will contribute to the development of electrochemical reactors and devices and the use of either of them will be determined by the individual needs of the system under investigation.
|
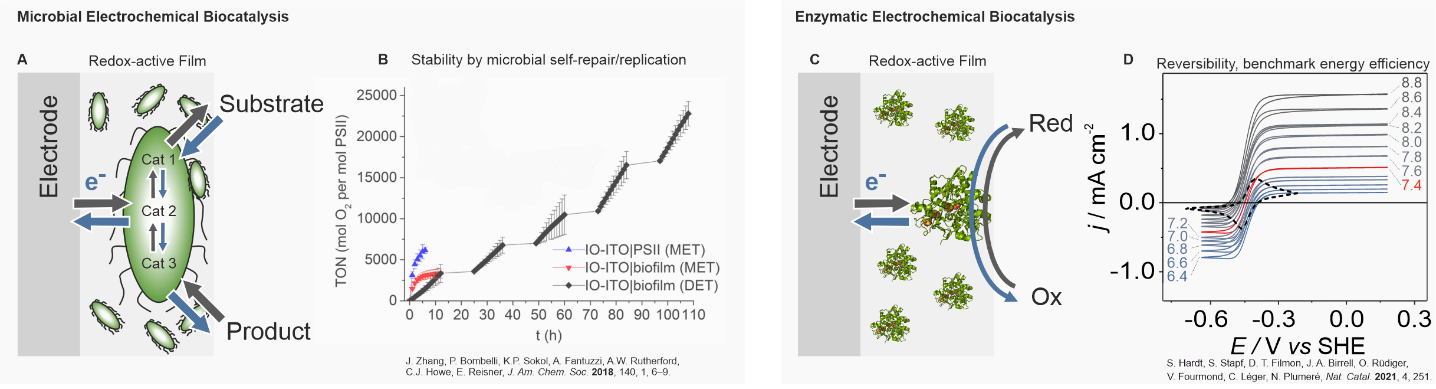
complex sequences or reactions (adapted from Ref 8). B. Representative example for the advantages of microbial electrochemical biocatalysis in terms of stability. A film consisting of photosynthetically active cyanobacteria (Synechocystis sp. PCC 6803 cells) remains active for photoelectrochemical oxygen evolution reaction for 110 h (black squares), whereas the isolated enzyme PSII degrades within less than 10 h (blue triangles).6 C. Schematic representation of a film embedding a highly selective redox-enzyme for the reversible interconversion of a reduced species (Red) to an oxidized species (Ox). The enzyme exchanges electrons with an external electrode. As a representative example the structure of [FeFe] hydrogenase from Desulfovibrio Desulfuricans was chosen. D. Example for the advantage of enzymatic bioelectrocatalysis with emphasis on the energy efficiency. Cyclic voltammetries show that [FeFe] hydrogenase embedded in a suitable polymer film catalyzes the interconversion of hydrogen and protons fully reversibly without a significant overpotential over a broad range of pH-values.3
References
(1) Chen, H.; Dong, F.; Minteer, S. D. The Progress and Outlook of Bioelectrocatalysis for the Production of Chemicals, Fuels and Materials. Nat Catal 2020, 3 (3), 225–244. DOI: 10.1038/s41929-019-0408-2.
(2) Lapinsonnière, L.; Picot, M.; Barrière, F. Enzymatic Versus Microbial Bio-Catalyzed Electrodes in Bio-Electrochemical Systems. ChemSusChem 2012, 5 (6), 995–1005. DOI: 10.1002/cssc.201100835.
(3) Hardt, S.; Stapf, S.; Filmon, D. T.; Birrell, J. A.; Rüdiger, O.; Fourmond, V.; Léger, C.; Plumeré, N. Reversible H2 Oxidation and Evolution by Hydrogenase Embedded in a Redox Polymer Film. Nat Catal 2021, 4 (3), 251–258. DOI: 10.1038/s41929-021-00586-1.
(4) Armstrong, F. A.; Hirst, J. Reversibility and efficiency in electrocatalytic energy conversion and lessons from enzymes. PNAS 2011, 34, 14049–14054. DOI: 10.1073/pnas.1103697108.
(5) Bollella, P.; Lo Gorton. Enzyme based amperometric biosensors. Curr. Opin. Electrochem. 2018, 10, 157–173. DOI: 10.1016/j.coelec.2018.06.003.
(6) Zhang, J. Z.; Bombelli, P.; Sokol, K. P.; Fantuzzi, A.; Rutherford, A. W.; Howe, C. J.; Reisner, E. Photoelectrochemistry of Photosystem II in Vitro vs in Vivo. J. Am. Chem. Soc. 2018, 140 (1), 6–9. DOI: 10.1021/jacs.7b08563.
(7) Kornienko, N.; Zhang, J. Z.; Sakimoto, K. K.; Yang, P.; Reisner, E. Interfacing Nature's Catalytic Machinery with Synthetic Materials for Semi-Artificial Photosynthesis. Nat. Nanotechnol. 2018, 13 (10), 890–899. DOI: 10.1038/s41565-018-0251-7.
(8) France, S. P.; Hepworth, L. J.; Turner, N. J.; Flitsch, S. L. Constructing Biocatalytic Cascades: In Vitro and in Vivo Approaches to de Novo Multi-Enzyme Pathways. ACS Catal. 2017, 7 (1), 710–724. DOI: 10.1021/acscatal.6b02979.
(9) Xiang, K.; Qiao, Y.; Ching, C. B.; Li, C. M. GldA Overexpressing-Engineered E. coli as Superior Electrocatalyst for Microbial Fuel Cells. Electrochem. Commun. 2009, 11 (8), 1593–1595. DOI: 10.1016/j.elecom.2009.06.004.
(10) Baikie, T. K.; Wey, L. T.; Lawrence, J. M.; Medipally, H.; Reisner, E.; Nowaczyk, M. M.; Friend, R. H.; Howe, C. J.; Schnedermann, C.; Rao, A.; Zhang, J. Z. Photosynthesis Re-wired on the Pico-Second Timescale. Nature 2023, 615 (7954), 836–840. DOI: 10.1038/s41586-023-05763-9.
|